About 2300 years ago, a human being measured the world. It was Eratosthenes, the Greek scholar. Imagine how he felt when he first realized what he had done. “Wow, I am the only person who has ever known the size of the world.” It must have been amazing. And humbling. He had noticed that sunlight falls at a different angle in Aswan than it does in Alexandria at the same time of day. That gave him a measurement of the world’s circumference accurate to within about 16%, an astounding achievement. Later, humans began measuring planets in our solar system beyond Earth, then planets around other stars.
It turns out we live on a medium-sized planet. It has enough gravity to hold a thick atmosphere, so air pressure keeps water in a liquid state at the surface. Air is needed to make fire so we could invent metallurgy. To support a technological civilization, a smaller world with only a subsurface ocean probably would not suffice. Other planets in our solar system are indeed much smaller than the Earth, but some are much, much larger. The range of sizes can be compared to the grains of sand in a bucket of lunar soil. You may find only one really big piece in there – a rock – and only a few pieces of gravel, but many, many sand grains and uncountable specks of dust. In terms of sheer numbers, nature tends to favor the small. The same is true in biology. There are only a few really large organisms on the Earth (whales, redwood trees, and gigantic underground fungi), but there are many more medium-sized organisms (people, lobsters, and carrots), huge numbers of insects, and countless microbes. The big ones are the rare cases, and the same is true for planetary bodies. Giants like Jupiter and Saturn are few. Medium-sized bodies like Earth and Pluto are more numerous. Smaller dwarf planets, asteroids and meteoroids are in vast supply.
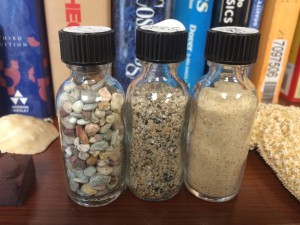
Three bottles from my sand collection. The size of the grains tells us the power of the waves on each one’s beach. Left to right, coarse to fine, from big waves to small.
Whenever we have a collection of objects like planets or sand, we like to study the distribution of their sizes because it gives insight into the dynamics of nature. I collect sand as a hobby. Whenever I travel I visit the local beaches or rivers to get samples of sand. (This leads to interesting conversations with airline security when they find baggies of the stuff in my luggage. But it’s surprising how many airport security agents love geology.) And sand collectors around the world exchange samples by mail. I’ve got hundreds of tiny bottles in all colors and textures – I find it truly fascinating, and it’s an excuse to spend more time in nature. On some beaches we see the sand is coarse, even gravelly, while on other beaches it is fine like silt. Why is this? The yearly power of the waves on each beach favor one type of sand grain over another. Big waves push coarse grains from the ocean floor up onto the beach but carry the smaller grains back out to sea. Small waves don’t have the energy to push coarser grains onto the shore nor to carry the really fine sand back out. The texture of the grains inside sandstone tell us what kind of waves, rivers, or winds collected the sand before it hardened into rock. In a similar way, biologists study the ecology of an island by measuring its animals. Are there a lot of big ones, and where do they get the food to maintain large body sizes? Do the carnivores tend to be bigger than the herbivores? And astrophysicists study the sizes of stars. Whether we are investigating biology, geology, or astrophysics, the distribution of sizes is an important clue.
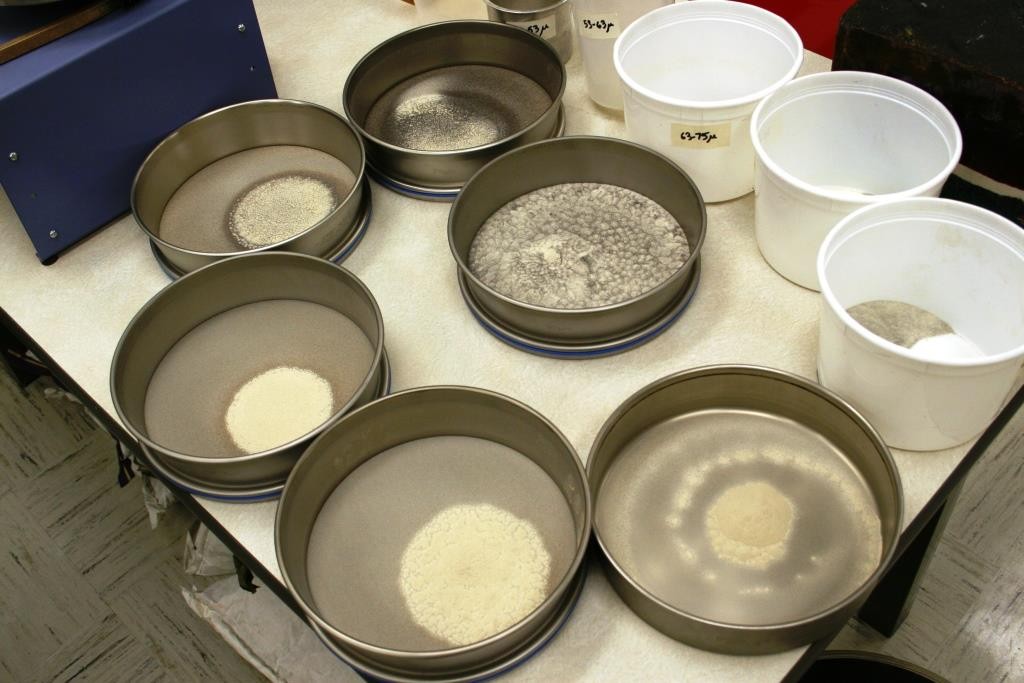
Sieving sand in my first lab at NASA. The sand runs down through a stack of screens to separate them into different sizes. I took this picture when I noticed the amazing sand patterns that formed on the screens.
On the Moon, sand grains are constantly broken down to smaller sizes by the impact of micrometeoroids (dust grains flying down from space at super-high velocity). These impacts don’t just break grains apart; they also melt some of the mineral, which splashes onto smaller sand grains and harden into glass, effectively gluing them together. This process of both breaking down and gluing together eventually reaches a steady state. We call lunar soil mature when it reaches that state. When a really big asteroid hits the Moon it digs deeply into the ground and blows out bedrock to make fresh, immature soil, which is very coarse. After sitting on the lunar surface for millions of years the micrometeoriod impacts make it mature so it displays the characteristic distribution of particle sizes, much finer than immature soil with lots of dust-sized particles. We study the age of lunar soil partly by looking at the distribution of particle sizes.
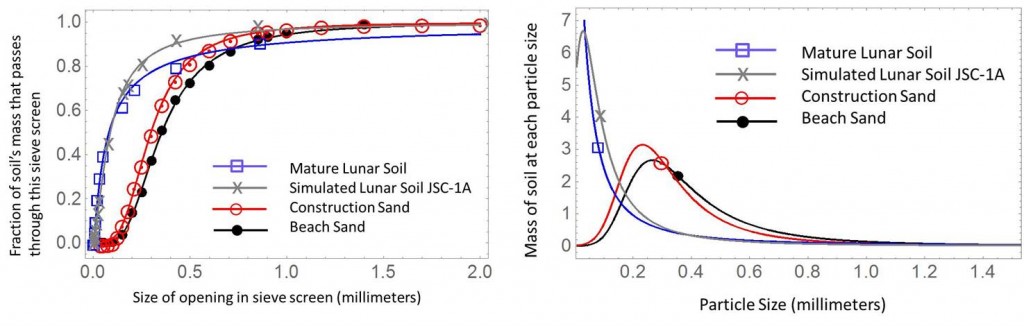
Left: The measured results from sieving four different granular materials. Right: After some math, the sieve results are converted into these plots that show how much of the soil’s mass consists of each particle size. Note that lunar soil (and the simulated lunar soil) have much smaller particles that beach sand or construction sand. Click to see larger plots.
What happens next to all this lunar dust? On the Earth, the dust is washed out of the soil by rain, and it is carried by streams and rivers into calm, shallow seas where it settles as mud. Over time, the mud hardens into mudstone and the rock cycle turns it into metamorphic or igneous rock. Thus, the geologic processes of Earth remove dust from the soil. Not so, on the Moon. There is no rain to remove the dust, so these tiny particles stay mixed into the soil everywhere on the Moon. Our bodies aren’t adapted to that geology so lunar dust is an engineering challenge. The particle sizes tell us about the geological processes that created them.
By the way, J.R.R. Tolkien’s story “Roverandom” tells of a dog who visits the Moon. He says the Moon is so clean, it is almost impossible to get dirty there. Ha!
We can do this kind of research with entire solar systems, too, where the planets are like grains of sand. We can ask questions such as, what astrophysical processes created this collection of planets? Why are the planets so much smaller in some regions of the solar system than in others? Why are there so many asteroids still floating around? Why are there so many medium-sized planets (dwarf planets) beyond Neptune? What solar system processes are building up or breaking down these bodies in each region?
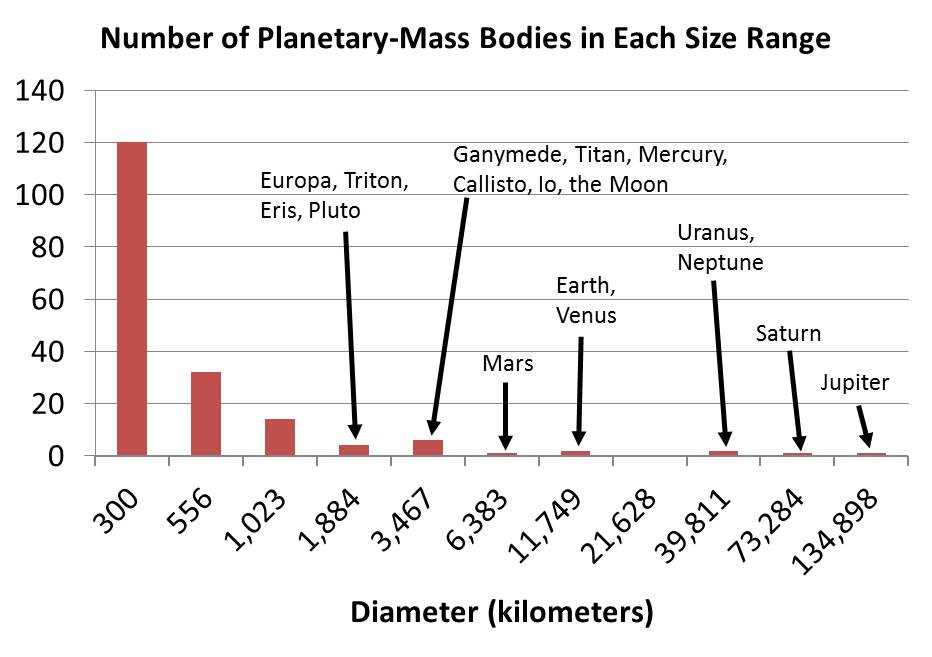
Nature favors smaller sizes among planets, too. Pluto and Earth are medium-sized planets in our solar system (using the geophysical definition of a planet). Moons are included as “secondary planets.”
We know planets grow by accretion. They start as dust grains orbiting the newly formed star, accumulating through electrostatics and gravity into planetesimals, colliding and growing further into planetoids, and eventually into the planets we see today. Most of this growth happens early in the solar system’s history until their orbits have been largely cleared. When there’s not much material for them left to absorb, the growth spurt necessarily ends. (Still, the Earth accretes about 60 tons of new mass from space every day, mostly in the form of dust.)
In the Main Asteroid Belt we see what looks like an anomaly: all that small stuff never grew into one bigger planet. We see the bodies are actually colliding with one another and busting apart instead of settling down into continual growth. It appears Jupiter is keeping the asteroid belt stirred up with its gravity so it never settles. The asteroid belt is the “beach” of the inner solar system, and Jupiter’s gravity is like waves crashing in from the sea. The particle sizes of this beach tell us something about the energy of the crashing waves. Is this process happening in lots of other solar systems, or do they have calmer seas and no asteroid belts? Or are the seas even rougher out there, so all the smaller planets are swept completely away? One day, when we send robotic probes to other stars to set up colonies in advance of humanity’s arrival, it would be very useful if they find some asteroid belts to mine. The natural place for a beachhead is a beach.
The Kuiper Belt out beyond Neptune is dynamically different than the Main Asteroid Belt. In recent decades we have been measuring the sizes of the Kuiper Belt Objects (KBOs). So far we have found only one example of a family of KBOs that broke apart by colliding. (In the asteroid belt we find many.) We call it the Haumea family because the dwarf planet Haumea appears to be the parent body. A long time ago, perhaps more than a billion years, another large body hit Haumea and blew out a dozen or more fragments. (Haumea’s two small moons are fragments that stayed trapped in Haumea’s gravity.) Scientists performed simulations of collisions in the Kuiper Belt and the Scattered Disc, and according to their results there is only a 47% chance that one such collision would occur. It seems likely, therefore, that the Haumea family is the only one. Unlike the Main Asteroid Belt, the planets aren’t dwarfed because collisions keep breaking them apart, but rather they are done growing because their orbits have been effectively cleared. In the inner solar system, bodies with intertwined orbits can’t coexist for very long (so we say those orbits aren’t cleared). In this third zone of the solar system, they can.
Planetary science is about to enter its heyday: we are collecting a vast wealth of data from the other solar systems in our corner of the Milky Way. It is still hard to measure the smaller planets in those systems, but our techniques and tools are improving so it’s just a matter of time (and of continued funding). This will revolutionize our understanding of solar system dynamics and will place our own solar system into a much larger context. It will help us fill in at least one unknown of the Drake Equation – how many life-supporting planets are out there? It will tell us the prospects for one day expanding our own civilization beyond Sol. It will let us know whether the Earth is unique, not just in our solar system, but in the universe. As T.S. Eliot said,
We shall not cease from exploration, and the end of all our exploring will be to arrive where we started and know the place for the first time.





